List members , one of the "myths" in Chemistry is that "organic compounds" or Carbon based compounds are only synthesised by living creatures and not independently in nature.
Now this old dogma of Chemistry , can be shattered by firm evidence of organic compounds continuously being synthesized in outer space . Not just that , Carbon in the form of C60 or the "buckminsterfullerenes" are also being found in outer space on a regular basis .
So , if C60 form of Carbon (a basic building block of Carbon chains essential for life) and even organic compounds are being synthesized "inorganically" in outer space , on a continuous basis , then why can't similar processes be occurring deep inside the Earth and other worlds ??
***The point is that there are INORGANIC processes in nature that create organic materials on a continuous basis , so abiotic oil from deep inside the Earth shouldn't come as a surprise !
https://royalsocietypublishing.org/doi/10.1098/rsta.2010.0231
The evolution of organic matter in space
Abstract
Carbon, and molecules made from it, have already been observed in the early Universe. During cosmic time, many galaxies undergo intense periods of star formation, during which heavy elements like carbon, oxygen, nitrogen, silicon and iron are produced. Also, many complex molecules, from carbon monoxide to polycyclic aromatic hydrocarbons, are detected in these systems, like they are for our own Galaxy. Interstellar molecular clouds and circumstellar envelopes are factories of complex molecular synthesis. A surprisingly high number of molecules that are used in contemporary biochemistry on the Earth are found in the interstellar medium, planetary atmospheres and surfaces, comets, asteroids and meteorites and interplanetary dust particles. Large quantities of extra-terrestrial material were delivered via comets and asteroids to young planetary surfaces during the heavy bombardment phase. Monitoring the formation and evolution of organic matter in space is crucial in order to determine the prebiotic reservoirs available to the early Earth. It is equally important to reveal abiotic routes to prebiotic molecules in the Earth environments. Materials from both carbon sources (extra-terrestrial and endogenous) may have contributed to biochemical pathways on the Earth leading to lifeās origin. The research avenues discussed also guide us to extend our knowledge to other habitable worlds.
1. The evolution of carbon in the Universe
The Universe is currently estimated to be approximately 13.7 Gyr old [1]. During Big-Bang nucleosynthesis, only H and He and traces of a few other light nuclei, such as D, T, Li and Be, were formed. Since the Universe was expanding, cooling and decreasing in density, heavier elements (called metals by astronomers) could not be formed during its early history, up to 500 Myr after the Big Bang. The triple-α process (3 Ć 4He ā 12C) in the cores of stars is the fundamental source of carbon, the basis of organic chemistry. After hydrogen is exhausted, further nuclear reactions start in the core of stars with masses greater than half a solar mass leading to carbon and heavier elements, and up to 56Fe for the more massive stars. Heavier elements than iron are formed during the final stages of stars in stellar explosions by neutron absorption ( s and r processes). In this phase, no equilibrium can be reached: the stars undergo mass loss either in a smooth way or in violent way, leading to the enrichment of the interstellar medium. Analytical arguments [2], as well as numerical simulations [3], showed that a significant amount of star formation could have occurred when the Universe was only 100 Myr old in the standard cold dark matter theory. The high opacity to Thomson scattering of the Universe found by the Wilkinson Microwave Anisotropy Probe provides support for this theory. The first stars (so called population III or zero metallicity stars) are expected to form in dark matter halos, growing from primordial fluctuations, in which the baryonic gas could cool efficiently enough. Although atomic cooling can ensure temperatures down to 10 000 K, molecular cooling is necessary to reach lower temperatures. The dominant cooling molecule at these stages is believed to be molecular hydrogen, H2, formed through H+eāāHā and Hā+HāH2+eā [4].
First stars are believed to be more massive than present day ones because H2 cooling could not decrease the temperature as low as metals do. The Jeans mass is therefore higher, up to 100ā1000 solar masses [5]. These massive stars are very short lived, with life times of less than 30 Myr and can no longer be observed. When they explode, large amounts of metals, in particular carbon and oxygen, are distributed. Subsequently, dust is synthesized and dispersed throughout the Universe [6]. There is evidence that a copious amount of molecules, such as O2, CO, SiS, SO, CO and SiO, may form in the ejecta of Pop III progenitor supernovae [7] that can be recycled in the next generation of forming stars. The recent detection of hyper metal-poor stars (HMPs) in the Milky Way halo also provides important constraints for early star formation processes [8,9]. The observed HMP stars have an Fe/H ratio less than 1/100 000 of the solar ratio. They have an overabundance in C and O relative to Fe, and are slightly less massive than the Sun. Their modest masses allow them to exist for longer than 10 Gyr, and thus to act as āfossilā record. Investigations of those stars may provide clues regarding the properties of the initial generation of stars and supernovae that distributed the first heavy elements in the early Universe. The star BD+44 493 has been recently identified as the brightest extremely low-metallicity star among all objects reported [10]. Carbon appears to be favoured as a formation product of the first generation of stars. Molecules like CO and water, formed once oxygen and carbon atoms were present (less than 1 Gyr after the Big Bang), as well as very small grains, cool interstellar gas much more efficiently than H2. This stimulates cloud fragmentation and triggers the formation of low-mass stars like our Sun [11ā14].
Low-mass stars live much longer, approximately 10 Gyr for the Sun, a duration that enables the formation of terrestrial planets and possibly the emergence of life.
Once the first generation of population III stars had formed and exploded as supernovae about 500 Myr after the Big Bang, the subsequent existence of metals and dust is expected to lead to the efficient formation of molecules like CO, water, hydrides and carbon chains [15]. Indeed, high excitation CO emissions from the distant quasar SDSS 114816.64+525150.3 at redshift z =6.4 have been detected, indicating that CO, one of the most important coolants of interstellar gas, has been present in the Universe since approximately 800 Myr after the Big Bang [16]. Furthermore, detections of C and C+ have been made and upper limits on HCN and water exist [17]. Somewhat more recently, at a redshift z =3.9, the gravitationally lensed quasar APM 08279 allows the inner 200 parsecs of the galaxy to be probed. Large amounts of warm (100 K) CO gas and warm dust (assuming a frequency squared absorption-coefficient dependence) appear to be present, indicative of active star formation [18]. Clearly, carbon and dust surface chemistry was occurring already in the earliest epochs of the Universe. That these objects with a significant amount of carbon are not flukes, is shown in figure 1, where the relative amount of carbon is presented as a function of redshift. In this, redshifts of 1 and 6 mean 35 and 7 per cent of the present age of the Universe, respectively. Clearly, carbon and thus star formation was already very much present in the young Universe [19]. This fits well with the star formation and metal-production history of the Universe [20], where the metals produced by massive stars lead to the formation of molecular gas and thus more stars. For a metallicity of 1 per cent of solar and up, one develops a multi-phase interstellar medium (ISM) that supports a cool dense phase, with a density larger than 100 cmā3 and a temperature less than 100 K, in which stars form and complex (carbon-chain) molecules can be produced [21]. Such a metallicity is certainly reached in the quasar sources mentioned above, and an active carbon chemistry therefore appears to have been in place for at least the past 10 Gyr.
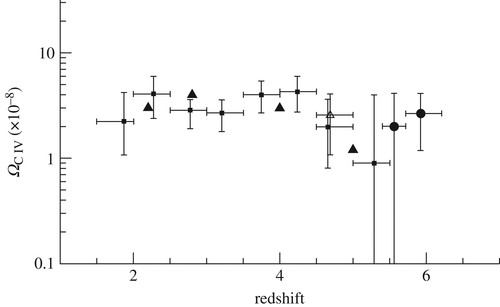
Figure 1. The evolution of the intergalactic ion C IV (C3+) abundance as a function of redshift, in units of its contribution to the closing density. The constancy of the curve indicates that vigorous star formation was already underway when the Universe was only 7% (at redshift z =6) of its present age. Reproduced with permission from Simcoe [19].
Closer to home, many if not all active galaxies are enjoying an active carbon chemistry, as exemplified by species such as CO, HCN, HNC, HCO+, CH3OH, H2CO, C2H and polycyclic aromatic hydrocarbons (PAHs; e.g. [22ā24]). Some galaxies form stars with a very high rate, so-called starburst galaxies like Arp 220 and M82. Carbonaceous molecules are detected in those systems as well, even in the presence of strong mechanical feedback owing to supernova explosions and outflows [25]. UV radiation produced by stars or X-ray photons created by an accreting supermassive black hole can destroy complex molecules in the nuclei of galaxies [26]. Nevertheless, species like CO may survive even such extreme environments, already in the early Universe at metal-enrichment levels of only a few per cent [27]. Also, PAHs have been detected with Spitzer out to high ( z >1) redshift (e.g. [28]). The cycle of birth and death of stars that was initiated by population III stars constantly increases the abundance of heavy elements in the interstellar medium, a crucial prerequisite for rocky-planet formation [29,30]. Understanding the early and active Universe is thus extremely relevant to the question of terrestrial-planet formation and consequently life.
2. The complexity of organic matter in space
(a) Interstellar clouds
Interstellar molecular clouds and circumstellar envelopes are factories of complex molecular synthesis [31ā33]. Interstellar clouds constitute a few per cent of galactic mass and are composed primarily of H and He. They are enriched primarily by matter ejected from evolved stars and can vary strongly in their fundamental physical parameters such as temperature and density. Dominated by gas, interstellar material also contains approximately 1 per cent of small micron-sized particles. Gas-phase and gasāgrain interactions lead to the formation of complex molecules. Surface catalysis on solid interstellar particles enables molecule formation and chemical pathways that cannot proceed in the gas phase owing to reaction barriers [34,35]. H2 is by far the most abundant molecule in cold interstellar regions, followed by CO, the most abundant carbon-containing species, with CO/H2ā¼10ā4. CO is characterized by a high binding energy (11.2 eV) and strongly influences the chemistry in interstellar clouds. Two main types of interstellar clouds drive molecular synthesis. In cold dark clouds, the temperature is low (approx. 10 K) and therefore the sticking coefficients of most atoms and molecules are close to unity, leading to a freeze out of practically all species (except H2 and He). The high density in dark clouds (up to approx. 106 cmā3) attenuates UV radiation and offers a protected environment for the formation of larger molecules by gas-phase reactions and ice chemistry on grains. A large number of complex organic gas-phase molecules was identified through infrared, radio, millimetre and sub-millimetre observations (http://www.astrochemistry.net lists more than 150 molecules). Among them are nitriles, aldehydes, alcohols, acids, ethers, ketones, amines and amides, as well as long-chain hydrocarbons. The main species observed in interstellar ice mantles are H2O, CO2, CO and CH3OH, with smaller admixtures of CH4, NH3, H2CO and HCOOH [36]. A recent c2d (core to disks) Spitzer survey of ices investigated the 6ā8 μm region that displays the prominent bending mode of water ice. Five independent components that can be attributed to eight different carriers [37] have been identified. Observations of CO2 in low-mass protostars showed high abundances (average of 32% relative to water ice) [38]. CH4 ice was detected in 25 out of 52 targets, with abundances ranging from 2 to 8 per cent relative to water ice and reach 13 per cent in a few sources [39].
Diffuse interstellar clouds are characterized by a low density (approx. 103 atoms cmā3) and temperatures less than or equal to 100 K. Ionāmolecule reactions, dissociative recombination with electrons, radiative association reactions and neutralāneutral reactions contribute to gas-phase processes and influence interstellar chemistry [40]. A variety of small molecules, including CO, CH, CN, OH, C2, C3 and others, are observed [41]. Gaseous PAHs are highly abundant, with fractions of about 10ā7 [42]. Carbonaceous grains composed of macromolecular aromatic networks account for most of the molecular carbon fraction in these clouds as evidenced by a ubiquitous strong UV absorption band at 2175 Ć
[43].
Amorphous carbon, hydrogenated amorphous carbon, diamonds, refractory organics and carbonaceous networks such as coal, soot, graphite and quenched-carbonaceous condensates have been proposed as possible carbon compounds [31,42,44,45]. PAHs are observed to be widely distributed in galactic and extragalactic regions [42,46ā49]. In diffuse interstellar clouds, dust interacts with hot gas, UV radiation and cosmic rays, and evolves or gets destroyed in shocks and by sputtering. Strong differences in the dust component of dense and diffuse interstellar clouds exclude rapid cycling of cloud material [50]. The formation pathway of solid carbonaceous matter is not yet understood. However, circumstellar envelopes are identified as factories of complex molecular synthesis [32]. Figure 2 shows a route to the formation of PAHs and soot material that has been proposed over two decades to occur in the shielded environment of stellar envelopes.
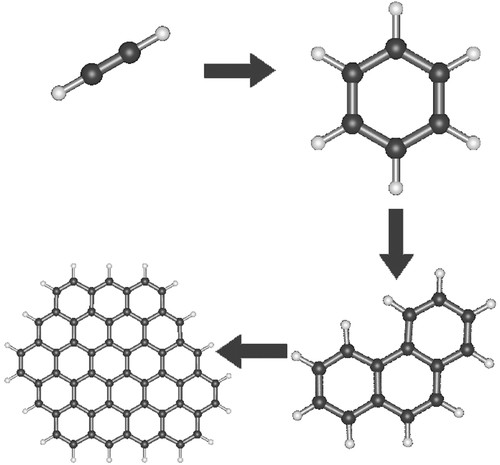
Figure 2. Acetylene chemistry leading to the formation of benzene and small PAHs that subsequently build up larger structures has been proposed to occur in the shielded environments of carbon-rich circumstellar envelopes (e.g. [32,51]). Aromatic materials, in solid and gaseous form, constitute the largest fraction of organic material in the Universe [52,53].
(b) Solar-System materials
The gravitational collapse of an interstellar cloud led to the formation of the protosolar nebula from which planets and small bodies of our Solar System formed [54]. Observations of protoplanetary disks show that gas and solids drift inward in time. Solids migrate inwards faster than gas, and small particles grow to kilometre-sized bodies that accrete to planets. The chemical composition of protoplanetary disks is expected to hold clues to the physical and chemical processes that influence the formation of planetary systems. Recently, it has been reported that the protoplanetary disks of AA Tauri possess a rich molecular emission spectrum in the mid-infrared, indicating a high abundance of simple organic molecules (HCN, C2H2 and CO2), water vapour and OH [55]. Data from recent space missions, such as the Spitzer telescope, Stardust and Deep Impact, show that the dynamic environment of the solar nebula with the simultaneous presence of gas, particles and energetic processes, including shock waves, lightning and radiation (e.g. [56]) can trigger a rich organic chemistry. Turbulent motion leads to radial mixing of the products within the disk [57,58], which has been confirmed by Stardust data [59]. The carbonaceous inventory of our Solar System therefore contains a mixture of material that was (i) highly processed by exposure to high temperatures and radiation, (ii) newly formed within the solar nebula, and (iii) captured as relatively pristine material with significant interstellar heritage.
Many organic compounds are observed or sampled from our Solar System, including planetary surfaces/atmospheres, comets and interplanetary dust [31,60ā62]. More than 50 molecules have been identified in cometary comae [63,64]. The analysis of carbon compounds in fragments of asteroid 2008 TC3 by Jenniskens et al. [65] recently revealed interesting insights into the asteroid chemistry. Carbonaceous chondrites (meteorites) and micrometeorites, which represent fragments of cometary and asteroidal bodies, do contain a variety of organics (e.g. see [62,66] for reviews).
In the soluble fraction of the Murchison meteorite, more than 70 extra-terrestrial amino acids have been identified in addition to many other organic compounds, including N-heterocycles, carboxylic acids, sulphonic and phosphonic acids, and aliphatic and aromatic hydrocarbons [67ā70]. However, the major carbon component in meteorite samples is composed of a macromolecular organic fraction [71]. A recent study using ultra-high resolution molecular analysis of the solvent-accessible organic fraction of Murchison shows high molecular diversity [72].
The remaining population of planetesimals not incorporated into planets exists until today as asteroids and comets. Most of the asteroids and comets are confined to stable orbits (such as the asteroid belt between Mars and Jupiter) or reservoirs in the outer Solar System (such as the Kuiper Belt) or beyond our Solar System (Oort cloud). In the early history of our Solar-System small bodies, such as comets and asteroids, and their fragments impacted the young planets [73]. A destabilized planetesimal disk caused a massive delivery of planetesimals to the inner Solar System at 3.9 Gyr ago. This so-called Late Heavy Bombardment (LHB) phase was likely triggered by rapid migration of giant planets [74]. The large quantities of extra-terrestrial material delivered to young terrestrial planetary surfaces during this period may have provided raw material useful for protocell assembly on the Earth [73,75]. The analysis of extra-terrestrial matter in the Earth laboratories indicates that the dominant form of carbonaceous material that was delivered to young planets must have been aromatic macromolecular matter.
3. Terrestrial planets
(a) Conditions on the early Earth
Planet Earth was formed through a hot accretion process about 4.6 Gyr ago, which allowed only the rocky material from the inner Solar System to survive. Although many interesting geological data of the early Earth were revealed in the last decade, we still have limited knowledge regarding the exact atmospheric composition, the temperature of the oceans and geological activity. Considerable geochemical evidence supports an initiation of plate tectonics on the Earth shortly after the end of the Hadean about 3.9 Gyr ago. It is unclear if the transition to plate tectonics resulted from radioactive decay-driven mantle heating or from cooling to the extent that large lithospheric plates stabilized [76]. Any existing carbon material from the solar nebula (volatile and refractory) was destroyed during the Earthās formation. Therefore, organic molecules found on terrestrial planets must have formed after the planetary surface cooled, or have been delivered via impacts by small bodies in the young Solar System. All terrestrial planets have been seeded with organic compounds through the impact of small bodies during Solar-System formation. There is a consensus that a combination of exogenous and endogenous sources has provided the first precursor molecules for life on the young Earth. These simple molecules reacted and assembled on catalytic surfaces (such as minerals) and formed more complex structures that later developed into primitive cells (protocells). The endogenous synthesis of prebiotic organic compounds (molecules that are involved in the processes leading to the origin of life) may have been limited to protective environments such as the oceans, owing to the hostile conditions on the young Earth, including volcanism, radiation and bombardment by comets and asteroids.
Whatever the inventory of endogenous organic compounds on the ancient Earth was, it would have been augmented by extra-terrestrial material. It is estimated that these sources delivered approximately 109 kg of carbon per year to the Earth during the heavy bombardment phase of 4.5ā3.9 Gyr ago [73].
Life on Earth is one of the outcomes of the formation and evolution of our Solar System and has adapted to every possible environment on planet Earth. Life on Earth can be found in extreme environments, such as hydrothermal vents, in dry deserts, the Earth atmosphere and in polar regions. These findings challenge the definition of the āplanetary habitable zoneā. Primitive life, in the form of bacteria, emerged approximately 3.5 Gyr ago [77,78]. The homologous series of long-chain aliphatic hydrocarbons found in old sedimentary rocks characterized by odd-over-even carbon-number predominance is only consistent with a biological origin and indicates that, despite the hostile conditions, planet Earth was habitable in its early history [79].
Today, the Earth provides an ideal environment for life to sustain. Dynamic processes in the interior of the Earth have established a magnetosphere that protects the Earth from harmful cosmic radiation. Oceans and atmosphere enable a stable climate and temperature cycle. Our neighbour planets are less fortunate. Venus, with an average surface temperature of 500°C and a steamy atmosphere, is unable to sustain life at its surface. Mars, with an average surface temperature of ā60°C and a thin toxic atmosphere, provides a hostile surface environment, but may harbour life in the subsurface.
Nitrogen is an essential element of both nucleotides (genetic code, energy transfer) and proteins. Systems containing NH3/NH+4 are more efficient in abiotic organic synthesis than those dominated by N2 in both aqueous and and gaseous environments [80]. The reason is that the triple bond between the atoms in molecular nitrogen is very stable and hard to break. Geochemically plausible abiotic synthesis pathways of prebiotic molecules and concentration mechanisms for nitrogen-containing molecules must eventually be found since nitrogen-based life is likely to have existed on the Earth from early Archean about 3.8 Gyr ago and onwards [81]. High ammonium contents (54ā95 ppm) have been found in authigenic clays of the Isua supracrustal rocks of western Greenland, suggesting that clays were major sinks of NH+4 or other nitrogen compounds on the Earthās surface already at 3.8 Gyr ago [82]. Ward & Brownlee [83] have argued that plate tectonics is necessary for the origin of life on terrestrial planets and have listed a number of reasons in support of their opinion. However, one argument that they have never mentioned is the connection between plate tectonics, hydrothermal geochemistry, and reduction of simple carbon and nitrogen compounds suitable for abiotic organic chemistry. The best location where such processes could occur would be at convergent margins during the early phases of subduction of oceanic plates. As a plate descends, fluids distilled from the plate influence, and even control, fundamental processes in the subduction zone [84]. The subducting plate, along with its fluids and altered igneous rock, interact with the over-riding plate along the subduction zone. Fluids originating in the subducting plate will rise through the upper plate containing hydrated ultramafic mantle material in its lower parts. The fluids may thus move from a relatively acidic environment in weathered basalt into an alkaline environment in serpentinized peridotite.
The strong pH increase promotes the formation of carbohydrates like ribose from simple organic compounds. It also supports the synthesis of amino acids and purine nitrogen bases, as well as their condensation to nucleotides in the presence of phosphorylated ribose.
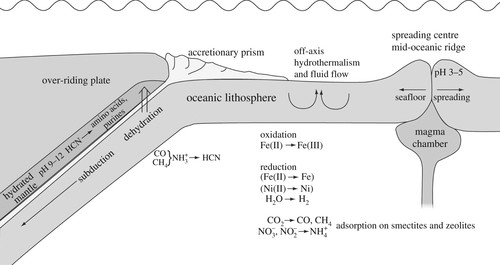
Figure 3. Cartoon showing a cross-section of oceanic lithosphere, extending from the spreading centre to the subduction zone. Off-axis hydrothermal flow in the oceanic lithosphere causes oxidation of Fe(II) to Fe(III) and reduction of water to molecular hydrogen. Some Fe(II) and Ni(II) is reduced to native metals. Carbon dioxide is reduced to carbon monoxide and methane, while nitrate and nitrite may be reduced to ammonium and adsorbed on secondary minerals like smectite and zeolites. During early subduction the descending plate is heated and dehydrated. Adsorbed carbon monoxide and methane may react with ammonia and form hydrogen cyanide. The released fluid carrying hydrogen cyanide rises from an environment of relatively low pH into hydrated mantle rock of high pH. At the high pH hydrogen cyanide may form HCN oligomers as well as amino acids, purine bases, nucleosides and, perhaps, nucleotides (adapted from [84]).
(b) Hydrothermal environments
Hydrogen cyanide is a key compound in abiotic synthesis of organic molecules. HCN is formed by a variety of processes driven by thermal energy, but is normally present in trace amounts [85]. HCN is, for instance, readily formed by the reaction of CO or CH4 with NH3 (figure 3) [84]. Hydrothermal systems represent regions of the highest conversion rates of nitrate and nitrite to ammonium on the Earth [86]. In general, the purine-coding elements of RNA (adenine and guanine) can be synthesized in the same abiotic reactions that yield amino acids [87ā90]. Adenine is likely to have been the first nucleobase formed abiotically, and has been shown to remain in detectable concentrations still after 200 h at 300°C under fugacities of CO2, N2 and H2 that are supposedly representative of those in marine hydrothermal systems on the early Earth [91]. The purines are formed from HCN via two routes. One route is via HCN oligomers, which may also form amino acids; the second one is via the HCN tetramer diaminomaleonitrile (DAMN) [87,88]. DAMN formation is accelerated by the presence of formaldehyde under mildly alkaline conditions (pH 9.2) [92]. Amino acids can be released by hydrolysis of HCN oligomers that form by the self-condensation of hydrogen cyanide in aqueous solution [88]. Furthermore, amino acids may be synthesized in putative prebiotic chemistries like Strecker-type reactions (synthesis of amino acids from cyanide and aldehyde in the presence of ammonia) in hydrothermal environments at fairly low temperatures (150°C) [93,94]. In order to participate in abiotic organic reactions, HCN must first be concentrated. One possibility is the concentration to a reservoir of ferrocyanide at relatively low pH from which free HCN can be released upon local elevation of the pH [81,95]. HCN reacts with ferrous ions to give ferrocyanide, provided that the concentration of hydrogen sulphide is low [96], like in the Lost City serpentinite-hosted hydrothermal field off the Mid-Atlantic Ridge [97]. The elevation of pH would lead to oxidation of Fe(II) and precipitation of the iron as FeOOH. This would avoid the āMiller paradoxā, which refers to the side reaction of glycolonitrile (cyanohydrin of formaldehyde) formation from free HCN and ubiquitous formaldehyde [98]. Formaldehyde and HCN, if present in the same environment, tend to react to form stable glyconitrile. On the other hand, Schwartz & Goverde [92] have shown that admixture of solutions enriched in cyanohydrins (like glyconitrile) with solutions of HCN permits oligomerization of HCN to DAMN, even under dilute conditions.
An alternative model to avoid the āMiller paradoxā involving alkaline hydrothermal mounds as flow reactors in which strongly polar compounds such as the cyanide ion is retained by fresh FeS/Fe3S4 membranes has been presented by Russell et al. [99ā102]. According to their model, the fluctuations in pH at the interface between hydrothermal fluid and sea water would determine adsorption and desorption of the cyanide. In natural environments, the occurrence of ferrocyanides in hydrothermal systems has so far been reported only from the Kurile Islands and the Kamchatka Peninsula in the northwest Pacific Ocean [103,104].
Cohn et al . [105] have shown that adenine is far displaced towards adsorption onto pyrite, quartz and pyrrhotite, which are all common minerals of hydrothermal environments. It would, therefore, normally be useless to search for the purine bases in the fluid phase of hydrothermal systems [106]. The observed coding effect of adsorbed purine bases [107] may indicate that the role of phosphate as a constituent of polynucleotides is a secondary property, and that phosphate was originally incorporated in prebiotic systems because of its energy-transfer capacity.
A couple of decades ago, many scientists believed that the abiotic formation of ribose, a constituent of RNA, occurred through the formose reaction [87,108]. The formose reaction was discovered and first described by Butlerow [109]. In this reaction, pentoses like ribose can be formed under alkaline conditions from simple organic precursors (formaldehyde and glycolaldehyde) [87,88]. The condensation of formaldehyde to sugars is catalysed by divalent cations and layered minerals, such as clays. The reaction proceeds by the stepwise condensation of formaldehyde to dimer (glycolaldehyde), trimer, etc. Under experimental conditions, it has been possible to convert as much as 50 per cent of the original formaldehyde to glycolaldehyde [110]. However, this reaction has, for a while, been an outdated concept in prebiotic chemistry. A major reason for this is that the reaction, as we have known it, is non-selective and leads to a large variety of aldoses, ketoses and sugar alcohols with only small fractions of potentially bioactive compounds such as ribose [90,111,112]. A general opinion has been that if ribose were used in the first RNA, an unknown selection process must have operated to segregate ribose from the other sugars that were formed. A second reason why the formose reaction has been outdated is that the reaction proceeds at a significant rate only under naturally āimprobableā conditions, like under highly alkaline conditions [81,87,108,113].
Natural environments with the pH conditions required for the abiotic formation of carbohydrates have previously been considered to be relatively rare on the Earth. However, the recent discovery of alkaline hydrothermal systems in ultramafic rocks, such as the Lost City Hydrothermal Field on the Mid-Atlantic Ridge [97,114ā116] and the Mariana Trench [94,84], indicates that alkaline environments may be much more common on the Earth than was thought just a few years ago.
Because of the postulated difference in requirements for the formation of the ribose and the nitrogen base, the spontaneous formation of RNA under prebiotic conditions on the Earth has been doubted [90]. The differences in required environment may, however, be illusive. It was mentioned earlier that formaldehyde is necessary for the formation of carbohydrates in the formose reaction. Schulte & Shock [117] have shown that aldehydes may be intermediates in the formation of carboxylic acids from hydrocarbons in sedimentary basin brines, as well as in hydrothermal systems. Furthermore, they concluded that the presence of aldehydes should normally be difficult to detect in natural systems if metastable equilibrium is reached between aldehydes and carboxylic acids at expected redox conditions. On the other hand, this suggests that aldehydes are always present as reaction intermediates in cases when organic acids and hydrocarbons both exist in natural hydrothermal systems. In fact, low concentrations of formaldehyde have been identified in hot-spring environments in Iceland, Mexico and southern California [118].
At equilibrium in hydrothermal systems, carboxylic acids, aldehydes and hydrocarbons will all be present in parallel, although at different levels of concentration.
4. Conclusion
The cycle of birth and death of stars that is initiated by population III stars constantly increases the abundance of heavy elements in the interstellar medium. Observations of the oldest stars show that carbon has been formed already in the very early Universe. The central star of our Solar System, the Sun, was formed many billion years after this time, and therefore contains several generations of supernova-produced metals. Tracing the earliest stars provides important insights into the origin of the elements necessary for life. The wide range of organic molecules identified by astronomical observations, space probes and by laboratory analysis of carbonaceous meteorites, suggests that the basic building blocks of life, at least as recognized on the Earth, must be widespread in planetary systems in our Galaxy and beyond [119]. There is a consensus that both exogenous and endogenous carbon sources have provided the raw material for the first building blocks of life on the early Earth.
The extra-terrestrial contribution arrived via comets, asteroids and small fragments during the LHB phase 3.9 Gyr ago. The large quantities of extra-terrestrial material delivered to young terrestrial planetary surfaces provided organic material predominantly in the form of aromatic networks that could have been used in biochemical pathways on the young Earth. Endogenous prebiotic carbon compounds may have formed in the early oceans. The connection between plate tectonics, hydrothermal geochemistry and reduction of simple carbon and nitrogen compounds has shown to be very suitable for abiotic organic chemistry. The best location where such processes could occur would be at convergent margins during the early phases of subduction of oceanic plates.
Understanding the early and active Universe is extremely relevant to the question of terrestrial-planet formation. How heavy elements assemble to complex molecules and evolve in interstellar and circumstellar regions and how they are incorporated into forming planetary systems and are used on young planets to form life are major research avenues that are investigated under the interdisciplinary umbrella of astrobiology. Understanding the constraints when defining the chemical composition of lifeās raw material from the viewpoints of different scientific disciplines is essential.
Acknowledgements
P.E. is supported by NASA grant NNX08AG78G and the NASA Astrobiology Institute NAI. We thank Alain Blanchard and Jan Cami for help in preparing the manuscript.
Research Article
Formation of buckminsterfullerene (C60) in interstellar space
Olivier BernƩ and A. G. G. M. Tielens
PNAS January 10, 2012 109 (2) 401-406; https://doi.org/10.1073/pnas.1114207108
- Edited by Neta A. Bahcall, Princeton University, Princeton, NJ, and approved November 2, 2011 (received for review August 31, 2011)
Abstract
Buckminsterfullerene (C60) was recently confirmed as the largest molecule identified in space. However, it remains unclear how and where this molecule is formed. It is generally believed that C60 is formed from the buildup of small carbonaceous compounds in the hot and dense envelopes of evolved stars. Analyzing infrared observations, obtained by Spitzer and Herschel, we found that C60 is efficiently formed in the tenuous and cold environment of an interstellar cloud illuminated by strong ultraviolet (UV) radiation fields. This implies that another formation pathway, efficient at low densities, must exist. Based on recent laboratory and theoretical studies, we argue that polycyclic aromatic hydrocarbons are converted into graphene, and subsequently C60, under UV irradiation from massive stars. This shows that alternativeātop-downāroutes are key to understanding the organic inventory in space.
The midinfrared spectra of a variety of astrophysical objects are dominated by band emission (strongest at 3.3, 6.2, 7.7, 8.6, and 11.2 μm) attributed to carbonaceous macromolecules [i.e., polycyclic aromatic hydrocarbons (PAHs)] (1). These molecules are large (30ā100 C atoms), abundant (approximately 5% of the elemental carbon), and their ionization plays a key role in the energy balance of gas in the interstellar medium (ISM) and in protoplanetary disk. In addition to PAH bands, infrared signatures observed at 7.0, 8.5, 17.4, and 19.0 μm have been reported recently (2, 3) and found to coincide precisely with the emission of buckminsterfullerene (C60) (4), a cage-like carbon molecule. This detection heralds the presence of a rich organic inventory and chemistry in space. However, observed abundances of C60 challenge the standard ion-molecule or grain-surface chemistry formation routes, which build up molecules from small to large in the ISM. For that reason, it has been suggested that C60 is formed in the hot and dense envelopes of evolved stars (5ā7) in processes similar to those found in sooty environments (8ā11), and eventually, is ejected in space. Yet, this scenario faces the problem that it has a limited efficiency (6). PAHs and C60 are known to coexist in the ISM (3); however, so far, the connection between PAHs and C60āand in particular the possibility to go from one compound to the other in spaceāhas not been investigated. In this paper, we present a study of PAH and C60 chemical evolution in the NGC 7023 nebula, using Spitzer (12) and Herschel (13) infrared observations.
Observational Results
Infrared Observations of the NGC 7023 Nebula.
Earlier Spitzer observations of the NGC 7023 reflection nebula have revealed a chemical evolution of PAHs: Deep in the cloud, emission is dominated by PAH clusters, which evaporate into free-flying PAHs when exposed to the UV radiation from the star (14ā16). There, gaseous PAHs are, in turn, ionized. While the neutral PAHs are dominated by zig-zag edgesāas demonstrated by the strong C-H solo out-of-plane modesāthe ions have an armchair molecular structure, characterized by strong duo out-of-plane modes (17). In regions closest to the star, the presence of C60 in the neutral state is evidenced by Spitzer observations (Fig. 1). New Herschel observations provide a measurement of dust emission in the same region, at high angular resolution (Fig. 1). This measurement can be used to derive the integrated intensity radiated by the nebula, which can be used as a calibrator, to convert the Spitzer observations of the PAHs and C60 bands into absolute chemical abundances of these species, allowing a quantitive study of PAH and C60 chemical evolution.
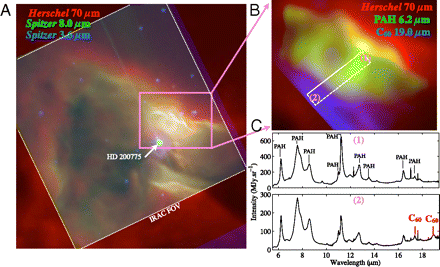
Fig. 1.
Overview of the NGC 7023 nebula. ( A ) Multiwavelength color-coded view of the nebula in the infrared. Red is the emission at 70 μm observed with the Photodetector Array Camera end Spectrometer (PACS) onboard Herschel. This emission is dominated by dust. Green is the Spitzer-Infrared Array Camera (IRAC) 8-μm emission tracing the PAH C-C mode, and blue is the IRAC 3.6-μm emission, tracing the PAH C-H mode and stellar emission. The position of the intermediate mass young star HD 200775 illuminating the nebula is indicated. ( B ) Color-coded image of the spatial distribution of different compounds in NGC 7023 : Red is the emission of dust observed with Herschel-PACS, green shows the emission integrated in the 6.2 μm C-C band of PAHs observed with Spitzer-Infrared Spectrograph (IRS), and blue is the C60 emission observed with Spitzer-IRS integrated in the 19.0-μm band. The white rectangle shows the region on which the extraction of the PAH and C60 abundance (Fig. 2) was performed. ( C ) Spitzer IRS midinfrared spectra taken at positions (1) ( Upper ) and (2) ( Lower ) in B . The distance from the star at positions (1) and (2) are, respectively, approximately 35ā²ā² and 15ā²ā². The bands of PAHs and C60 are labeled in the spectra.
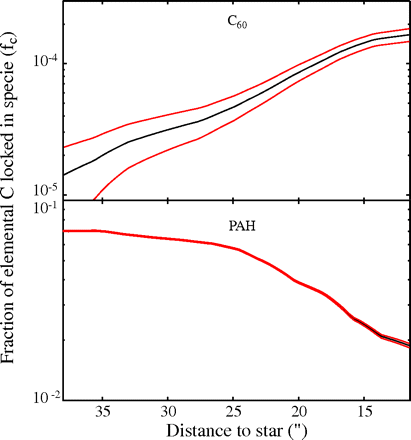
Fig. 2.
Abundances of C60 ( A ) and PAHs ( B ) in NGC 7023 as a function of distance from the star in the cut shown in Fig. 1. The red curves give the 1 sigma uncertainty, obtained from the propagation of instrumental uncertainty on the extraction of integrated intensities of PAHs and C60 bands.
Measurement of the Far Infrared Integrated Intensity of Dust Emission in the Nebula.
The far infrared integrated intensity I FIR was extracted by fitting the spectral energy distribution (SED) at each position in the cross-cut shown in Fig. 1. For these positions, we have used the brightnesses as measured by Herschel Photodetector Array Camera end Spectrometer (PACS) (18) and SPIRE (19) photometers. We have used the 70- and 160-μm channels of PACS and the 250-μm channel of SPIRE. This data is presented in detail in ref. 20. The modified blackbody function fit to these SEDs containing three spectral points is defined by
[1]where K is a scaling parameter, λ is the spectral index, and B ( λ , T ) is the Planck function with λ the wavelength and T the temperature. We have used a constant value of 1.8 for β so that only T and K are free parameters. The results of the fits to the observations are shown in Fig. S1. The temperatures we have derived from the fit of the data range between 25 and 30 K. These values are in agreement with those of ref. 20 for the same region. The peaking position of the modified blackbody function moves to shorter wavelengths (i.e., the grain temperature increases) when getting closer to the star, implying that we are indeed tracing matter inside the cavity and not material behind on the line of sight. The far infrared integrated intensity, I FIR, is then derived by integrating I ( λ , T ) over frequencies.
Measurement of the Integrated Intensity of PAH and C60 Emission in the Nebula.
The integrated intensity of PAH emission, I PAH, is measured by fitting a PAH emission model to the observed Spitzer midinfrared low-resolution spectrum. This data covers the 5- to 14-μm range where most of the emission occurs. Because this spectral range contains the emission due to the vibration of both C-C and C-H bonds, it is insensitive to ionization of PAHs. An example of this fitting model is presented in ref. 21. This tool provides the integrated intensity in the PAH bands as an output and takes care of continuum subtraction and extinction correction. The C60 integrated intensity ( I C60) extraction needs to be done separately for each band. We first measure the integrated intensity in the 19- and 17.4-μm band. The first step consists in extracting the intensity in the 19.0-μm band ( I 19.0) by fitting a Gaussian and subtracting a local linear continuum. The 17.4-μm band is contaminated by PAH emission, but this can be removed effectively. The contribution of PAHs to the 17.4-μm band can be estimated in this way: In the outer regions of the nebula, the 17.4-μm band is 100% due to PAHs (because no C60 is detected there) and so is the 16.4-μm band. The 16.4- and 17.4-μm band of PAHs are known to correlate. Indeed, in the outer regions of the nebula we find
. Therefore, over the whole nebula, we can estimate the intensity of the 17.4-μm band due to C60 by
. Fig. S2 shows the result of this process on the map. The 7.0-μm band is faint and usually hard to detect. In the regions closest to the star where both the 19- and 7.0-μm features are observed, we can calibrate the ratio of I 19.0/ I 7.0. It is found to be relatively stable (at least in this small zone close to the star) and of the order of 0.4. Therefore, we use I 7.0 = 0.4 à I 19.0. The 8.5-μm band is undetectable because of the strong PAH band present at 8.6 μm, so we use the ratio provided in ref. 3 for 5 eV photons; that is, I 8.5 = 0.4 à I 19.0. The total C60 IR emission is hence given by I C 60 = I 19.0 + I 17.4 - I 16.4 à 0.35 + I 19.0 à 0.4 + I 19.0 à 0.4.
Derivation of PAH and C60 Abundances.
The method to derive the abundance of carbon locked in PAHs and C60 from I PAH, I C60, and I FIR is presented in detail in ref. 22. The fraction of carbon locked in PAHs and C60 (respectively,
and
) per atom of interstellar hydrogen are given by
[2]and
[3]where
[4]and
[5]
and
are the UV absorption cross-sections of PAHs and C60. Following ref. 22, we adopt
per C atom. There are no detailed measurements of the UV absorption cross-section of C60; following ref. 3, we adopt the same value as for PAHs.
Evidence of C60 Formation in NGC 7023.
The results of the abundance variations within the nebula as derived using the above method are shown in Fig. 2. The C60 abundance in the nebula is seen to increase from 1.4 Ć 10-4 to 1.7 Ć 10-2% of the elemental carbon abundance when approaching the star (Fig. 2). On the other hand, the abundance of PAHs is seen to decrease, from 7.0 to 1.8% of the carbon (Fig. 2). This shows that C60 is being formed in the nebula while PAHs are being destroyed or processed. The correlation of these variations with the increasing UV field strongly suggests that UV photons control C60 formation and PAH processing and destruction.
The Top-Down Model
Proposed Scenario for the Formation of C60 in the ISM.
The formation of C60 in the ISM is unexpected and has several implications in our understanding of the formation process of this molecule. In the laboratory, the main formation route invoked for C60 is the buildup from atomic carbon, small carbon clusters or rings (8ā11). In space, such processes are efficient in the hot (1,500 K) and dense ( n H > 1011 cm-3) envelopes of evolved stars (6). In NGC 7023, the gas is approximately seven orders of magnitude less dense (see ref. 23 and SI Text ), making this aggregation processes inefficient. Instead, we invoke photochemical processing of PAHs as an important route to form C60. Upon UV irradiation, several channels for fragmentation can be open depending on excitation energy; e.g., H loss and C2H2 loss (24). However, experiments on small PAHs have shown that H loss is by far the dominant channel (25) and that complete dehydrogenation [i.e., graphene ([26](https://www.pnas.org/content/109/2/401#ref-26)) formation] is then the outcome of the UV photolysis process (27, 22, 28, 29). In a second step, carbon loss followed by pentagon formation initiates the curling of the molecule (30). We envision that this is followed by migration of the pentagons within the molecule, leading to the zipping-up of the open edges forming the closed fullerene (31) (Fig. 3 and Movie S1). Graphene formation through PAH photolysis can give rise to a rich chemistry. Besides the route toward fullerenes outlined above, fragmentation toward small cages, rings, and chains may also result. The relative importance of isomerization and fragmentation will determine the carbon inventory delivered by the photochemical evolution of PAHs in space (Fig. 3).
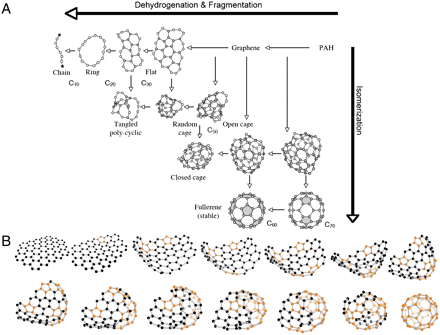
Fig. 3.
Schematic representation of top-down interstellar carbon chemistry. ( A ) The chemical evolution of PAHs in the ISM under the influence of UV photons combines the effects of dehydrogenation and fragmentation with those of isomerization. Fully hydrogenated PAHsāinjected by stars into the ISMāare at the top right side. Near bright stars, UV photolysis will preferentially lead to complete H loss (e.g., the āweakest linkā) and the formation of graphene. Further fragmentation may lead to the formation of flats, rings, and chains. However, this process competes with isomerization to various types of stable intermediaries such as cages and fullerenes. ( B ) Schematic illustration of conversion of graphene into C60 in 13 steps. Dehydrogenated PAHs (i.e., graphene sheets) loose carbon atoms under UV irradiation, giving rise to pentagonal defects (represented with orange C atoms) at the edges of the sheet. These defects in the hexagonal network induce curvature of the sheet, the migration of the pentagons allows the molecule to close. Image courtesy of L. Cadars (www.laurecadars.com).
PAH and Graphene Stability in Space.
Guided by experimental (27) and theoretical (28) studies, we have evaluated the stability of PAHs against dehydrogenation, and C60 formation, in conditions appropriate for NGC 7023.
Graphene Formation
Schematically, the fragmentation process can be written as
[6]where PAHā is the excited species that can stabilize through emission of IR photons or through fragmentation and PAH-H is a dehydrogenated PAH radical. There are various ways to evaluate the unimolecular dissociation rate constant for this process (compare ref. 22, section 6.4). We will follow ref. 32 and write the rate constant in Arrhenius form,
[7]where T e is an effective excitation temperature, E o is the Arrhenius energy describing the process, and the preexponential factor k o depends on the interaction potential (in the reverse reaction). For PAHs, the internal excitation of the vibrational modes after the absorption of a UV photon can be well described by
[8]where N c is the number of C atoms and E is internal energy in eV (ref. 22, p. 184). Because typically the energy involved in these reactions is a fair fraction of the total energy in the system, a correction has to be made to this excitation temperature. The finite heat bath correction results in (22, 32)
[9]The preexponential factor can be set equal to
[10]with Ī S the entropy change for which we will adopt 5 cal/K (28). k o is then, typically, ā3 Ć 1016 s-1. The Arrhenius energy parameter, E o , cannot be easily evaluated from theoretical calculations (33). Here, we use a fit to the experimental fragmentation studies on small PAHs [< 24 C atoms; (25)], which results in E o = 3.3 eV (ref. 22, p. 204). The probability for dissociation depends then on the competition between fragmentation and IR photon emission,
[11]where k ir ( E ) is the IR emission rate at an internal energy E . For a highly excited PAH, k ir ( E ) is approximately 1 s-1. The total fragmentation rate is then
[12]where k uv( E ) is the absorption rate of UV photons with energy, E .
The photochemically driven H loss is balanced by reactions of atomic hydrogen with dehydrogenated PAHs; namely,
[13]The rate of this reaction has been measured to be k a = 1.5 Ć 10-10 cm3 s-1 for a number of small PAHs (28, 34). We can define the dissociation parameter,
. With k uv = 7 Ć 10-10 N c G o , where G o is the flux of UV photons in units of the interstellar Habing field (35), we have Ļ ā 0.2 n H / N c G o p d ( E ). The hydrogen coverage of interstellar PAHs is a very sensitive function of Ļ , and a small increase of Ļ can change PAHs from fully hydrogenated to graphene (22, 34). This is illustrated for the circumovalene, C60H20, in Fig. S3. Thus, PAHs are fully hydrogenated if Ļ is much less than 1 and fully dehydrogenated if Ļ is much larger than 1. Hence,
[14]provide a critical relation for the transformation of PAHs into graphene. We have evaluated this relation assuming an absorbed UV photon energy of 10 eV (Fig. 4).
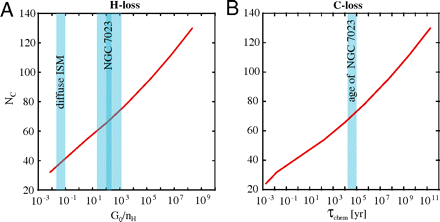
Fig. 4.
Photochemistry of PAHs in NGC 7023 ( A ) The red curve shows the evolution of the critical value when PAHs have lost half of their H atoms, as a function of the physical conditions ( G 0/ n H where G 0 is the radiation field in Habing units and n H is the density of H atoms in the gas in cm-3; see SI Text for details) and the number of carbon atoms in the PAH molecule N C . Above this line, PAHs are stable against dehydrogenation. Below this curve, PAHs rapidly loose all their H atoms. The value of G 0/ n H for NGC 7023 is shown; dark blue represents the ānarrowā range, and clear blue represents the ābroadā range of values for this parameter ( SI Text ). ( B ) Timescale for the loss of a carbon atom by UV photon absorption as a function of graphene sheet size.
Fullerene Formation
After formation of graphene, UV photoabsorption can lead to loss of carbon from the skeleton. This fragmentation process competes with stabilization through IR photon emission. The reaction rate is again given by Eq. 7 . We adopt Ī S = 5 cal/K, resulting in k o ā 3 Ć 10-16 s-1, but the exact value is not critical. For E o , we have adopted the Arrhenius energy [3.65 eV (36)] derived from experiments on the C loss from small catacondensed PAHs (25). The calculated cohesive energy of carbon in graphene is much larger, 7.4 eV per C atom (37) but that refers to typically carbon inside the skeleton, and carbon at the edge will be less strongly bound. Ref. 31 finds a theoretical value of 5.4 eV for the C atoms at a zig-zag edge. Moreover, theoretical cohesive energies are not a good measure for the Arrhenius energy in unimolecular dissociation experiments (33). Given the low abundance of gas phase carbon, the reverse reaction is unimportant and the chemical lifetime [
] has to be compared to the dynamical lifetime of the region. We have evaluated this chemical lifetime for a typical internal energy of 10 eV as a function of the size of the graphene sheet, N c , and the results are shown in Fig. 4.
Application to NGC 7023.
To evaluate graphene formation in NGC 7023, we look at PAH stability. The physical conditions in the nebula, characterized by parameters G 0 and n H , can be obtained ( SI Text ). As shown in Fig. 4, H loss is very efficient in NGC 7023 for PAHs up to 70 C atoms. We then evaluate the timescale for the loss of C atoms by the graphene flakes and compare this to the dynamical age of the nebula for a ā70 C atom PAH (Fig. 4). It appears that if we adopt E o = 3.65 eV, C loss is rapid compared to the age of the nebula.
Discussion
Our models reveal that 70 C atoms PAH become unstable to graphene formation in the studied region of NGC 7023. Much larger PAHs will survive closer to the star, whereas smaller PAHs will rapidly be completely dehydrogenated further away, butābecause of sizeāthis will not lead to the formation of fullerenes. As shown in laboratory experiments (31), graphene sheets larger than about 70 C atoms can be transformed into fullerene, but in space this is driven by UV photons rather than energetic electrons. We surmise that fullerene formation is initiated by single C atom loss (38) at the zig-zag edge of the graphene flake. Quantum chemical calculations indeed show that the armchair structure of graphene clusters stabilizes through in plane Ļ -bond formation, whereas the open-shell orbitals associated with the dangling bonds are located at the zig-zag edge (37), making these atoms more labile (31). A detailed study of graphene edge structure (39), based on experimental results (38), has indeed shown that single C atom loss leads to the formation of reconstructed edges bearing pentagons. These defects dramatically stress graphene flakes, inducing significant positive curvature in its topology (30). Eventually, the barrierless migration of the pentagons within the hexagonal network will lead to the zipping-up of the flake into C60 (31) (Fig. 3). The above mechanism is severely limited by the physical conditions, controlling dehydrogenation, and the size of the precursors, which need to be above approximately 70 C atoms. As shown by our observations, only about 1% of the available PAHs are converted into C60 by this process (Fig. 2). The activation of this ātop-downā chemistry requires the high UV fields available near massive stars. As a corollary, high abundance of C60 in the diffuse ISM (40) reflects processing by massive stars, which are capable of dehydrogenating PAHs in the relevant size range (60ā70 C atoms). PAHs much larger than than 70 C atoms will survive even in close encounters with massive stars. Smaller-size PAHs (< 50 C atoms) cannot reach the fullerene island of stability, hence their photoprocessing (Fig. 2) must be a source of various carbon nanocompounds (45) (e.g., rings, chains, cages, bowls, tubes, etc.) (Fig. 3). Laboratory experiments have indeed shown that processing of small graphene constrictions can lead to carbon rings and chains (41). Finally, we note that recent studies suggest that PAH molecules do not provide a satisfactory explanation to the diffuse interstellar bands (42, 43). The compounds described in this top-down chemistry may be relevant to understand these features of the UV extinction curve, in the Milky Way and in other galaxies (44).
Conclusion
Analyzing infrared observations of the NGC 7023 nebula, we have found evidence that C60 is being formed in the ISM. Classical bottom-up formation routes fail to explain these observations, so we propose a chemical route in which C60 is formed directly by the photochemical processing of large PAH molecules. Other carbonaceous compounds (cages, tubes, bowls) are also the product of this photoprocessing. This route must be relevant in the ISM, but may also be important in the inner regions of protoplanetary disks around solar-type stars where accretion on the young star generates intense UV fields and PAHs are known to be present. There, they can be converted, on timescale of approximately 1 million years, into fullerenes and hydrocarbon fragments. This source of organic compounds remains to be considered in models studying the organic photochemistry of the ISM and regions of terrestrial planet formation. It is clear that such studies may benefit greatly from the progress achieved in the field of graphene stability.
Acknowledgments
The authors thank the referees for their constructive comments. Laure Cadars (www.laurecadars.com) is acknowledged for producing the C60 formation sketch. Studies of interstellar PAHs at Leiden Observatory are supported through advanced European Research Council Grant 246976.
Footnotes
-
āµ1To whom correspondence should be addressed. E-mail: [email protected].
-
Author contributions: O.B. performed research; A.G.G.M.T. performed modeling; A.G.G.M.T. wrote section on dehydrogenation; O.B. analyzed data; and O.B. wrote the paper.
-
The authors declare no conflict of interest.
-
This article is a PNAS Direct Submission.
-
This article contains supporting information online at www.pnas.org/lookup/suppl/doi:10.1073/pnas.1114207108/-/DCSupplemental.
Regards